Optogenetics Guide
Introduction
The field of optogenetics integrates optics and genetic engineering to measure and manipulate cells (frequently neurons) and their governing biomolecular processes. The tools and technologies developed for optogenetics research utilize light to detect, measure, and control molecular signals and cells in order to understand their functions.
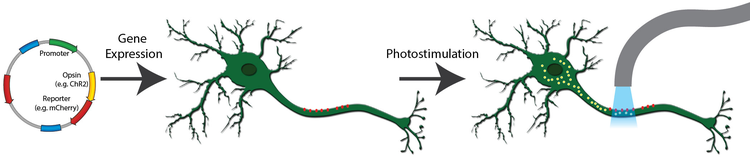
Example schematic of an optogenetics procedure. A channelrhodopsin, fused to mCherry, is expressed in neurons (red dots). When exposed to light of the correct wavelength, the pore opens, cations flow into the cell (yellow dots), and the neuron is activated.
Optogenetics tools can be broadly classified based on their functions into two groups:
- Actuators are genetically-encoded tools for light-activated control of proteins; e.g., microbial opsins and optical switches
- Sensors are genetically-encoded reporters of molecular signals; e.g., calcium indicators
In this overview, we will focus on the common actuators used in optogenetics. For information on sensors, check out our Biosensors Plasmid Collection.
To find optogenetics plasmids available through Addgene, check out our Optogenetics Plasmid Collection.
Microbial Opsins
Opsins are light-gated ion channels or pumps that absorb light at specific wavelengths. Upon activation by light, these channels and pumps respond by opening or closing, which conducts the flow of ions into or out of the cell. Scientists have identified a variety of naturally occurring microbial opsins that respond to different wavelengths of light, like blue or yellow light. These various opsins also initiate different electrochemical responses, such as nonspecific cation influx vs. proton efflux. Researchers have used genetic engineering to improve these natural opsins - by inducing point mutations to alter the absorption spectrum or adding trafficking signals to localize opsins to the cell membrane.
Microbial opsins, such as those described below, can be targeted and expressed in specific subsets of neurons, allowing precise spatiotemporal control of these neurons by turning on and off the light source. Optogenetics has been broadly applied to study the physiology of the brain and nervous system to better map and understand neuronal circuits. Optogenetic tools have also been used to trigger and study certain behavioral responses in model organisms like mice, zebrafish, and Drosophila. These tools have been instrumental in neurological disorder research, helping scientists to better understand Parkinson’s disease, depression, drug addiction, and more.
Below is a list of commonly used microbial opsins, including a brief overview of the wild-type opsin of each and some examples of relevant variants. This list is not exhaustive - please browse our curated list of opsins here to find the right optogenetics plasmids for your experiments.
Channelrhodopsins
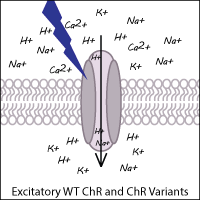
Channelrhodopsins are a foundational optogenetics tool - they typically allow the fast depolarization of neurons upon exposure to light through direct stimulation of ion channels. Naturally occurring channelrhodopsins were discovered in the green algae Chlamydomonas reinhardtii. Channelrhodopsin-1 (ChR1) is excited by blue light and permits nonspecific cation influx into the cell when stimulated. Channelrhodopsin-2 (ChR2), the first widely adopted optogenetic tool, is also a blue light activated cation channel. ChR2 is preferred over ChR1 because ChR2 has higher conductance at physiological pH and trafficks well to the membrane.
-
Excitatory (Depolarizing) ChR Variants. The optogenetic toolbox has been expanded by scientists through both the identification of novel ChRs from other algal species and the development of synthetic variants to enhance the functionality of ChR. Examples of ChRs from other species include: CsChR (from Chloromonas subdivisa), CoChR (from Chloromonas oogama), and SdChR (from Scherffelia dubia). Synthetic variants have been created via genetic point mutations, codon optimization, and chimeric fusion of domains from two different ChRs. These ChR variants still function as light-gated, cation channels resulting in excitation (depolarization) of the neuron. Feature enhancements include:
- Increased photocurrent amplitude
- Examples: ChR2(H134R), C1V1(t/t), ChIEF
- Increased channel (on/off) kinetics:
- Examples: ChETA, C1V1(t/t), ChrimsonR
- Red-shifted peak action spectra
- Examples: VChR1, C1V1(t/t), Chrimson, ChrimsonR, Chronos
- Increased photocurrent amplitude
-
Inhibitory (Hyperpolarizing) ChR Variants. Alternatively, ChR variants that inhibit neurons have been created and identified in other species - by acting as light-gated chloride channels, these variants result in the hyperpolarization of neurons. Examples of anion channel variants from other species include: GtACR1 and GtACR2 (from the cryptophyte Guillardia theta). Other feature enhancements include:
- Increased photocurrent amplitude
- Examples: iChloC, SwiChRca, Phobos, Aurora
- Increased photocurrent amplitude
Browse Channelrhodospin plasmids.
Halorhodopsins
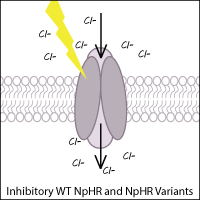
Halorhodopsins are light-gated inward chloride pumps isolated from halobacteria. Wild-type halorhodopsin, known as NpHR (from Natronomonas pharaoni), causes hyperpolarization (inhibition) of the cell when triggered with yellow light, thus inhibiting function of the neuron.
-
NpHR Variants. Variants have been engineered with enhancements such as:
- Human codon optimization
- Example: Halo
- Increased photocurrent amplitude
- Examples: eNpHR, eNpHR2.0, eNpHR3.0
- Red-shifted peak action spectra
- Example: Jaws
- Human codon optimization
Browse Halorhodopsin plasmids.
Archaerhodopsins
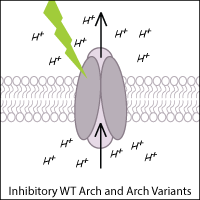
Archaerhodopsin-3 (Arch) from Halorubrum sodomense is also commonly used to inhibit neurons in optogenetic experiments. Arch is a light-activated outward proton pump that hyperpolarizes (inhibits) the cell when triggered by green-yellow light.
-
Arch Variants. Arch variants have been developed with the following enhancements:
-
Increased light sensitivity
- Example: ArchT
- Increased photocurrent amplitude
- Examples: eArch3.0, eArchT3.0
-
Increased light sensitivity
Browse Archaerhodopsin plasmids.
Wild-Type Mac
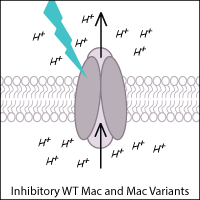
Leptosphaeria rhodopsin (Mac) is a blue-green light-activated proton pump derived from the fungus Leptosphaeria maculans. Mac and its variants allow for inhibition of neurons using blue-green light.
-
Mac Variants. Mac variants have been engineered to include enhancements such as:
-
Improved photocurrent amplitude
- Example: eMac3.0
-
Improved photocurrent amplitude
Browse Leptosphaeria plasmids.
Glossary of Microbial Opsin Variants
Opsin Type | Variant | Description | Peak Response Spectra (nm) |
---|---|---|---|
Channelrhodopsins: cation channels from Chlamydomonas reinhardtii | ChR2 | Widely used light-gated cation channel from Chlamydomonas reinhardtii (CrChR2) | 470 |
ChR2/H134 | Widely used variant with larger photocurrent compared to CrChR2 | 450 | |
ChETA | E123T mutation; creates faster kinetics but reduces photocurrent amplitude | 490 | |
ChR/T159C | T159C mutation; displays increased photocurrents | 470 | |
SFO/SSFO | Step function Opsins and Stabilized Step Function Opsins. Mutations at C128 and D156 of CrChR delay the closing of the channel | 470(activ.), 590(inact.) | |
ReaChR | Red-activatable variant of CrChR2 | 590 | |
Channelrhodopsins: cation channels from other species | VChR1 | Channelrhodopsin from Volvox carteri | 570 |
Chronos | High-speed, light-sensitive channel from Stigeoclonium helveticum | 500 | |
Chrimson, ChrimsonR | Red-light drivable channel from Chlamydomonas noctigama. ChrimsonR carries K176R mutation. | 590 | |
PsChR2 | High-efficiency blue-shifted channelrhodopsin from Platymonas subcordiformis | 445 | |
CoChR | Channelrhodopsin from Chloromonas oogama | ||
CsChR | Channelrhodopsin from Chloromonas subdivisa | ||
CheRiff | Channelrhodopsin from Scherffelia dubia | ||
Channelrhodopsins: cation channels- chimeras | C1C2 | ChR1-ChR2 chimera | 470 |
ChIEF, ChEF, ChD | Engineered by chimeragenesis of ChR1 and ChR2 | 450-470 | |
C1V1 | ChR1-VChR1 chimera with E122T and E162T mutations | 540 | |
Channelrhodopsins: chloride channels | iChloC | Chloride-conducting channel, CrChR2 with mutations E90R, D156N, T159C, CE83Q & E101S | 465 |
SwiChRca | Chloride-conducting channel, Step-waveform inhibitory ChR with mutation C128A (derived from C1C2 chimera) | 475 | |
GtACR | Chloride-conducting channel from Guillardia theta | 515(ACR1), 470(ACR2) | |
PsChR1 | Red-shifted chloride-conducting channel from Proteomonas sulcata | 540 | |
Phobos | Blue-shifted iC++ variant | 467 | |
Aurora | Red-shifted anion-selective ReaChR variant | 517 | |
Halorhodopsins | Jaws | Red-shifted, light-driven inward chloride pump from Haloarcula (Halobacterium) salinarum (strain Shark), used to silence neuronal activity | 632 |
Halo/NpHR | Light-driven inward chloride pump from Natronomonas pharaonis, used to silence neuronal activity | 589 | |
eNpHR 3.0 | NpHR with addition of trafficking signal from Kir2.1 and ER export signal provide improved membrane targeting | 589 | |
Archaerhodopsins | Arch | Light-activated outward proton pump from Halorubrum sodomense | 566 |
eArch 3.0 | Arch with addition of trafficking signal from Kir2.1 | 566 | |
ArchT | Light-activated outward proton pump from Halorubrum strain TP009. Improved light sensitivity over Arch. | 566 | |
eArchT 3.0 | ArchT with addition of trafficking signal from Kir2.1 | 566 | |
Leptosphaeria rhodopsins | Mac | Light-activated outward proton pump from Leptosphaeria maculans | 540 |
eMac 3.0 | Mac with addition of trafficking signal from Kir2.1 | 540 |
Plan Your Optogenetics Experiment
When designing your optogenetics experiment, you’ll need to pick both an opsin and a delivery system. Here are some key factors to consider:
Optogenetic excitation or optogenetic inhibition. First things first: do you want to turn ON or turn OFF neurons in your experiment? Depending on your answer, you’d pick an excitatory or inhibitory opsin, respectively.
Color of activation light. There are a variety of different activation wavelengths, ranging from blue to yellow to red. Red light exhibits better tissue penetrance, which may allow you to place the optic fiber outside of the brain, rendering the experimental procedure less invasive. Different activation wavelengths also make it possible to combine multiple opsins in the same experiment. For example, you could use different colors of light to activate/silence the same neuronal population, or activate different neural populations at distinct times.
Temporal considerations. Temporal precision is key in optogenetic experiments. Your experimental design will determine whether you’ll need short or long periods of neuronal activation/inactivation periods. These can range from msec (hChR2) to “long lasting,” e.g. seconds to minutes with stable step-function opsins (SSFOs).
Opsin delivery systems. Two factors determine which neuronal population is manipulated in a given experiment: the expression of the opsin and the area that is being illuminated. There are several different ways to control opsin expression. Generally, the most robust and stable expression is achieved in a transgenic mouse line, such as for example the VGAT-ChR2 mouse, where ChR2 is expressed under the control of the vesicular gamma aminobutyric acid (GABA) transporter (VGAT) and ChR2 is thus expressed in all GABAergic neurons. In this case, the subpopulation of GABAergic neurons being activated by the light is controlled via the placement of the optic fiber.
Using viral vectors for opsin delivery results in more localized expression of the opsin. Depending on the virus and promoter system used, there is an incubation time (days to weeks) until peak expression of the opsin is reached. This approach is especially powerful when combined with site specific recombinase technology like Cre-lox, which allows you to express an opsin ONLY in a genetically defined subpopulation of cells within the injection site of the viral vector, rather than in all cells. For example, using a viral vector with a floxed opsin in a VGAT-cre animal will result in expression of the opsin only in inhibitory neurons near the injection site.
Optical Switches


Researchers have harnessed plant and bacterial photoreceptors to create protein systems controlled by light. These “photoswitchable” proteins offer exquisite spatial and temporal control of protein activity. Phytochrome, cryptochrome, and LOV (light oxygen voltage)-based systems have been used in many experimental contexts, including protein activation, membrane localization, and transcriptional activation.
In the widely used Cry2-CIB1 system, light induces a conformational change in cryptochrome Cry2 to permit CIB1 binding. As shown in the figure above, this light induced binding can be utilized to control the localization of a protein of interest. A genetically-encoded nuclear Cry2 fusion can thus direct a CIB1-protein fusion to the nucleus upon light activiation. Alternatively, when one half of a given protein, such as the Cre recombinase, is fused to Cry2 and the other half to CIB1, light-stimulated heterodimerization can reconstitute the protein. This principle has also permitted the design of synthetic two-part transcription factors - Cry2 and CIB1 are fused to a transcriptional activation domain and DNA binding domain, allowing light to activate transcription.
Phytochromes and light oxygen voltage (LOV) domains function similarly to cryptochromes with light-induced changes in protein conformation/dimerization. In addition to the applications described above, both LOV and Dronpa can control activity of a fused protein through allosteric interactions. Researchers continue to engineer new optobiology tools and improve upon previous tools through directed mutagenesis - use Addgene's Optical Switch Plasmid Table to search for plasmids for your next experiment. For a full description of different domains used in these plasmids, see the Glossary below.
Glossary of Optical Switches
Category | Variant | Description | Peak Response Spectra (nm) |
---|---|---|---|
BLUF domains | bPAC | Light-activated adenylyl cyclase from Beggiatoa sp. for light-induced cAMP modulation. Utilizes BLUF (blue light utilizing FAD) domains. | 441 |
Phytochromes | BphP1–PpsR2 | Bacterial phytochrome BphP1 and its natural partner PpsR2 from Rhodopseudomonas palustris bacteria. Key feature is the sensitivity to 740- to 780-nm near-infrared light. | 740–780 |
Phy–PIF | Light-controlled Phytochrome Interacting Factor 6 (PIF6) to PhyB (Phy) interaction | 660 | |
Cryptochromes | Cryptochrome 2 and CIB1 | Blue-light–mediated induction of protein interactions based on Arabidopsis thaliana cryptochrome 2 and CIB1 | 450 |
LARIAT | Inhibits protein function by reversibly sequestering targets into large, optically assembled protein architectures in living mammalian cells. | ||
LITE | Two-hybrid system integrating the customizable TALE DNA-binding domain with cryptochrome 2 protein and its interacting partner CIB1 to control transcription effectors | ||
OPTOSTIM | PHR domain2 of cryptochrome 2 (Cry2) from Arabidopsis thaliana is fused to truncated forms of cytosolic STIM1 to control calcium channels | ~488 | |
LOV domains | LOV-based | Light-oxygen-voltage-sensing (LOV) domains are protein sensors used by many plants, microalgae, fungi and bacteria to sense environmental conditions. These domains are fused to different effector domains of proteins to generate novel engineered light-controlled molecules. | ~450–495 |
BACCS | light-activated calcium channel switch | ||
iLID | Improved light-inducible dimers; Bacterial SsrA peptide is embedded in the LOV2 domain (iLID plasmid), while its natural binding partner SspB is included in a separate plasmid; Blue light activation induces a conformational change in the LOV2 domain which allows SsrA to bind with SspB | ||
LAD | Light-activated dimerization, using proteins GIGANTEA (GI) and the light oxygen voltage (LOV) domain of FKF1 | ||
LITEZ | Light-inducible transcription using engineered zinc finger proteins, using proteins GIGANTEA (GI) and the light oxygen voltage (LOV) domain of FKF1 | ||
LINUS | light-inducible nuclear localization signal based on the LOV2 domain of Avena sativa phototropin 1 | ||
LOVETRAP | reversibly sequester and release proteins from cellular membranes using light. light-induced protein dissociation using protein A fragments (called Zdk) that bind to the LOV domain only in the dark. attaching only one member of the Zdk/LOV2 pair to the target protein, and the other to the membrane where the target protein is to be sequestered | ||
Lumitoxin | Peptide toxin for blocking K + (Kv) channels fused to LOV2 photoswitch. Illumination causes the photoswitch to unfold, lowering the toxin's local concentration near the cell surface, and enabling the ion channel to function. | ||
TULIPs | Tunable light-inducible dimerization tags (TULIPs), plasmids allow cloning of protein coding sequences with GFP-LOVpep, cpPDZ, ePDZb and ePDZb1 as tags. | ||
pDawn/pDusk | Blue-light photoreceptor for light-controlled gene expression. These plasmids rely on the engineered two-component regulatory system YF1/FixJ. YF1 is a synthetic, photosensitive kinase, which uses the ubiquitous flavin mononucleotide as its chromophore, and phosphorylates the transcriptional activator FixJ in the absence of blue light. | 470 | |
Fluorescent Protein domains | Dronpa based | Light-inducible Dronpa mutant domains that associate and cage a protein in the dark, while dissociate and activate the protein in the light | ~400 (on)/~500 (off) |
PhoCl | Photocleavable protein engineered from a green-to-red photoconvertible fluorescent protein | 400 | |
UVR8 domains | UVR8 | plant photoreceptor protein that forms photolabile homodimers with slow reversal kinetics and a UV-B absorption profile | 282 |
References
Optogenetic Switches from OptoBase, W. Weber Lab
Edward Boyden's Lab: Synthetic Neurobiology Group Optogenetics Resources
Karl Deisseroth's Lab: Optogenetics Resource Center
Boyden ES, Zhang F, Bamberg E, Nagel G, Deisseroth K. 2005. Millisecond-timescale, genetically targeted optical control of neural activity. Nat Neurosci. 8(9):1263-8. PMID 16116447
Chuong AS, Miri ML, Busskamp V, Matthews GA, Acker LC, Sørensen AT, Young A, Klapoetke NC, Henninger MA, Kodandaramaiah SB, et al. 2014. Noninvasive optical inhibition with a red-shifted microbial rhodopsin. Nat Neurosci. 17(8):1123-9. PMID 24997763
Deisseroth K, Feng G, Majewska AK, Miesenböck G, Ting A, Schnitzer MJ. 2006. Next-generation optical technologies for illuminating genetically targeted brain circuits. J Neurosci. 26(41):10380-6. PMID 17035522
Dugué GP, Akemann W, Knöpfel T. A comprehensive concept of optogenetics. 2012 Prog Brain Res. 196: 1-28. PMID 22341318
Gradinaru V, Zhang F, Ramakrishnan C, Mattis J, Prakash R, Diester I, Goshen I, Thompson KR, Deisseroth K. 2010 Molecular and cellular approaches for diversifying and extending optogenetics. Cell. 196:1-28. PMID 20303157
Han X, Boyden ES. 2007 Multiple-color optical activation, silencing, and desynchronization of neural activity, with single-spike temporal resolution. PLoS One. 2(3):e299. PMID 17375185
Kim B, Lin MZ. 2013. Optobiology: optical control of biological processes via protein engineering. Biochem Soc Trans. 41(5): 1183–1188. PMID 24059506
Klapoetke NC, Murata Y, Kim SS, Pulver SR, Birdsey-Benson A, Cho YK, Morimoto TK, Chuong AS, Carpenter EJ, Tian Z, et al. 2014. Independent optical excitation of distinct neural populations. Nat Methods. 11(3):338-46. PMID 24509633
Lin, J. 2011. A User’s Guide to Channelrhodopsin Variants: Features, Limitations and Future Developments. Exp Physiol. 96(1): 19–25. PMID 20621963
Mattis J, Tye KM, Ferenczi EA, Ramakrishnan C, O'Shea DJ, Prakash R, Gunaydin LA, Hyun M, Fenno LE, Gradinaru V, Yizhar O, Deisseroth K. 2011. Principles for applying optogenetic tools derived from direct comparative analysis of microbial opsins. Nat Methods. 9(2):159-72. PMID 22179551
Saunders A, Johnson CA, Sabatini BL 2012. Novel recombinant adeno-associated viruses for Cre activated and inactivated transgene expression in neurons. Front Neural Circuits. 6:47. PMID 22866029
Wietek J, Beltramo R, Scanziani M, Hegemann P, Oertner TG, Wiegert JS. 2015. An improved chloride-conducting channelrhodopsin for light-induced inhibition of neuronal activity in vivo. Sci Rep. 5:14807. PMID 26443033
Wietek J, Prigge M. 2016. Enhancing Channelrhodopsins: An Overview. Methods Mol Biol. 1408:141-65. PMID 26965121
Yizhar O, Fenno L, Zhang F, Hegemann P, Diesseroth K. 2011. Microbial opsins: a family of single-component tools for optical control of neural activity. Cold Spring Harb Protoc. 3:top102. PMID 21363959
Yizhar O, Fenno LE, Prigge M, Schneider F, Davidson TJ, O'Shea DJ, Sohal VS, Goshen I, Finkelstein J, Paz JT, et al. 2011. Neocortical excitation/inhibition balance in information processing and social dysfunction. Nature. 477(7363):171-8. PMID 21796121
Zhang F, Prigge M, Beyrière F, Tsunoda SP, Mattis J, Yizhar O, Hegemann P, Deisseroth K. 2008. Red-shifted optogenetic excitation: a tool for fast neural control derived from Volvox carteri. Nat Neurosci. 11(6):631-3. PMID 18432196